Review Article - Journal of Drug and Alcohol Research ( 2023) Volume 12, Issue 5
Progressive Innovations in Advanced Functional Materials for Emerging Bio-electronics, Drugs Sensing and Healthcare
Iqra Qayyum1,2*, Fazal Ur Rehman1,2, Manzar Zahra1,3, Kanwal Batool4, Waseem Shoukat5, Sabeen Arshad6, Shahid Anwar7, Aoun Raza1 and Zeshan Zada82Department of Chemistry, University of Education, Pakistan
3Department of Chemistry, Quaid-e-Azam University, Pakistan
4Department of Chemistry, Minhaj University, Pakistan
5Institute of Chemical Sciences, Bahauddin Zakariya University, Multan-60800, Pakistan
6Department of Nanoscience, Rovira I Virgili University, Tarragona, Spain
7Research and Development Department, CCL Pharmaceuticals, Pakistan
8Department of Physics and Molecular Modelling, Islamia College Peshawar, KPK, Pakistan
Iqra Qayyum, Department of Chemistry, Lahore Garrison University, Pakistan, Email: iqraqayyum6@gmail.com
Received: 31-May-2023, Manuscript No. JDAR-23-107658 ; Editor assigned: 02-Jun-2023, Pre QC No. JDAR-23-107658 (PQ); Reviewed: 16-Jun-2023, QC No. JDAR-23-107658 ; Revised: 21-Jun-2023, Manuscript No. JDAR-23-107658 (R); Published: 28-Jun-2023, DOI: 10.4303/JDAR/236244
Abstract
In recent years, materials science has seen remarkable advancements, yielding novel functional materials applied in various industries, including biology, healthcare, and bioelectronics. This communication paper highlights advanced functional materials’ significant contributions in biological and bio-electronic applications. It showcases drug delivery systems, tissue engineering scaffolds, biosensors, bio-imaging agents, neural interfaces, and wearable health monitoring devices. In medicine, these materials enable targeted and sustained drug release, transforming treatments for diseases. In tissue engineering, they promote tissue regeneration and repair. Enhanced biosensor sensitivity and selectivity enable rapid disease detection. In bioelectronics, materials like conducting polymers, graphene, and nanocomposites facilitate neural interface applications and wearable health monitoring devices. Despite challenges, the future prospects are promising, revolutionizing healthcare with personalized therapies and advancing regenerative medicine. These materials bridge materials science and biology, unlocking possibilities for the future.
Keywords
Advanced functional materials; Bioelectronic applications; Drug delivery systems; Tissue engineering scaffolds; Biosensors
Introduction
The field of bio-electronics is rapidly evolving, thanks to the development of advanced functional materials [1]. These materials have unique properties that make them ideal for medical applications, such as diagnostics, treatment, and wearable healthcare technologies. One of the most promising applications of advanced functional materials in healthcare is the development of more accurate and sensitive medical diagnostics. For example, carbon-based nanomaterials can be used to create ultrasensitive biosensors that can detect even minute amounts of biomarkers in blood or other body fluids [2]. This could lead to earlier diagnosis of diseases, which could improve patient outcomes.
Another promising application of advanced functional materials in healthcare is the development of new treatment modalities for diseases [3]. For example, smart hydrogels can be used to deliver drugs or genes directly to target cells in the body. This could lead to more effective and targeted treatments for diseases [4].
In addition to diagnostics and treatment, advanced functional materials are also being used to develop wearable healthcare devices that can monitor and track health data. These devices could be used to track heart rate, blood pressure, blood sugar levels, and other vital signs [5]. This information could be used to identify early signs of disease or to provide feedback on a patient’s adherence to a treatment plan.
The development of advanced functional materials for bio-electronics and healthcare is a rapidly growing field with the potential to revolutionize the way we diagnose, treat, and manage diseases [6]. These materials are opening up new possibilities for personalized medicine and could lead to improved patient outcomes.
Drug and Alcohol Sensing Research
Advanced functional materials have been at the forefront of research in various fields, including drug and alcohol sensing. These materials offer unique properties and functionalities that make them well-suited for detecting and quantifying specific substances. Some of the advanced functional materials commonly used in drug and alcohol sensing applications include: Carbon nanotubes are cylindrical structures composed of carbon atoms. They possess excellent electrical conductivity and a high surface area, making them suitable for sensing applications. CNT-based sensors can be functionalized with specific receptors to detect and quantify target drugs or alcohol molecules with high sensitivity.
Graphene is a single layer of carbon atoms arranged in a two-dimensional honeycomb lattice. It exhibits exceptional electrical and mechanical properties, making it a promising material for sensors. Functionalized graphene sensors have been explored for their ability to detect drugs and alcohol at low concentrations due to their high surface area and sensitivity.
Metal-Organic Frameworks (MOFs) are porous materials composed of metal ions coordinated with organic ligands. Their tunable structure allows for the incorporation of different functional groups, enabling selective detection of specific substances. MOFs have shown promise in sensing drugs and alcohol with high selectivity and sensitivity. Conducting polymers are organic materials that exhibit electrical conductivity upon doping. These materials can be designed to interact with specific drug or alcohol molecules, leading to changes in electrical properties that can be detected and quantified.
Quantum Dots (QDs) are semiconductor nanocrystals with unique electronic properties. They can be engineered to emit light of specific wavelengths upon excitation. Functionalizing QDs with receptors allows for the detection of target substances based on fluorescence changes, enabling sensitive and selective sensing.
Metal nanoparticles, such as gold and silver nanoparticles, have shown potential in drug and alcohol sensing applications. These nanoparticles can be modified with specific receptors, and their optical properties can be used to monitor changes upon interaction with target substances.
Nanowires and nanorods
Semiconductor nanowires and nanorods have been investigated for drug and alcohol sensing due to their high surface-to-volume ratio and unique electronic properties. These materials can be functionalized with various receptors to detect specific substances.
2D Transition Metal Dichalcogenides (TMDs) such as molybdenum disulfide and tungsten disulfide, are layered materials with intriguing electronic properties. Functionalizing TMDs with specific ligands can enable the detection of drugs and alcohol molecules with high sensitivity.
In drug and alcohol sensing, the choice of material depends on factors such as sensitivity, selectivity, response time, and stability. Researchers continue to explore and develop novel functional materials to improve the performance of sensors for accurate and reliable detection of drugs and alcohol in various applications, including law enforcement, healthcare, and public safety.
Drug Delivery Systems
Advanced functional materials have ushered in a new era of drug delivery strategies, revolutionizing the field of medicine (Figure 1). Among these materials, nanoparticles, liposomes, and hydrogels have emerged as the frontrunners, offering a plethora of benefits that have transformed the way drugs are administered and their efficacy in treating diseases [7].
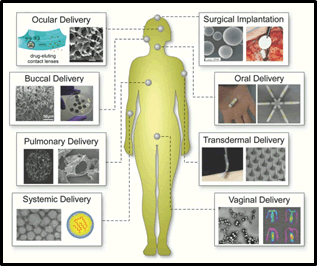
Figure 1: Different types of drug delivery being used by advance functional materials
Nanoparticles, with their nanoscale size, have exceptional properties that make them ideal carriers for drug delivery. They can encapsulate drugs, protecting them from degradation and enhancing their stability. Moreover, their small size allows for improved circulation in the bloodstream, facilitating their delivery to specific target sites in the body. This targeted delivery minimizes exposure to healthy tissues and maximizes drug accumulation at the disease site, resulting in enhanced therapeutic outcomes [8].
Liposomes, on the other hand, are specialized vesicles composed of lipids that can encapsulate both hydrophilic and hydrophobic drugs (Figure 2). These versatile carriers offer a unique advantage by accommodating a wide range of therapeutic agents [9]. Liposomes have the ability to fuse with cell membranes, facilitating the direct release of drugs into target cells. This mechanism allows for controlled and sustained drug release, leading to prolonged therapeutic effects and reduced dosing frequency [10].
Smart hydrogels represent another class of advanced functional materials that have played a transformative role in drug delivery [11]. These three-dimensional networks of hydrophilic polymers can absorb and retain large amounts of water. The incorporation of drug molecules into hydrogels enables controlled release in response to specific stimuli, such as changes in temperature, pH, or enzyme concentration (Figure 3) [12]. This responsiveness ensures that drugs are released at the desired location or under specific physiological conditions, further enhancing the precision of drug delivery [13].
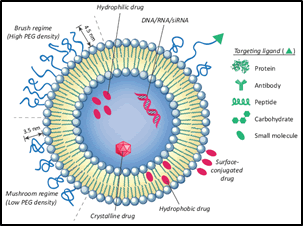
Figure 2: Liposomes as potential drug carrier systems for drug delivery
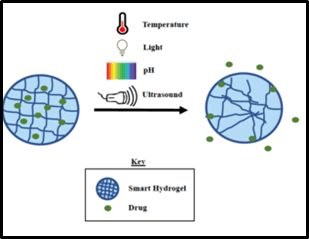
Figure 3: Stimuli responsive based hydrogels
One of the most significant advantages of advanced functional materials is their ability to reduce the toxicity associated with conventional drug delivery methods [14]. By selectively targeting the disease site, these materials minimize off-target effects, sparing healthy tissues from unnecessary exposure to potent drugs. This reduction in toxicity not only improves patient comfort but also increases the overall safety profile of the treatment.
Furthermore, the precise control over drug dosage offered by functional materials is a game-changer in the field of medicine. Tailoring drug release profiles ensures that patients receive the right amount of medication at the right time, optimizing therapeutic benefits and minimizing adverse effects. This level of precision allows for personalized treatment plans, catering to individual patient needs and disease characteristics.
Tissue Engineering Scaffolds
Functional materials play a pivotal role in the field of tissue engineering, serving as essential scaffolds that provide a three-dimensional (3D) framework for cell growth and tissue regeneration (Figure 4) [15]. These materials, ranging from electrospun nanofibers to hydrogels and decellularized matrices, offer a biocompatible and biodegradable platform that closely mimics the natural microenvironment of living tissues. By creating a biomimetic environment, functional scaffolds facilitate crucial processes such as cell adhesion, proliferation, and differentiation, ultimately fostering successful tissue regeneration [16].
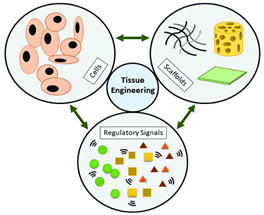
Figure 4: Tissue engineering applications
Electrospun nanofibers are among the remarkable functional materials extensively used in tissue engineering [17]. These ultra-fine fibers, often in the nanometer scale, can be fabricated from biocompatible polymers and provide an intricate, porous structure. This unique architecture enhances the surface area available for cell attachment and nutrient exchange, creating an optimal environment for cells to thrive and regenerate tissues. Hydrogels, on the other hand, are three-dimensional networks of hydrophilic polymers that can absorb and retain water [18]. These materials possess tunable physical properties, such as mechanical strength and porosity, making them suitable candidates for a wide range of tissue engineering applications. Their soft and gel-like consistency closely resembles the extracellular matrix, supporting cellular infiltration and tissue growth.
Decellularized matrices represent another class of functional materials that have gained prominence in tissue engineering [19]. Derived from natural tissues or organs, these matrices are stripped of cellular components, leaving behind a scaffold rich in bioactive molecules and structural proteins. By providing a natural architecture and chemical cues, decellularized matrices promote cell adhesion and guide tissue-specific differentiation, making them an excellent substrate for regenerating complex tissues.
The true potential of functional materials as scaffolds lies in their ability to be precisely tailored to match the requirements of specific tissues [20]. The functionalization of scaffolds with growth factors, bioactive molecules, and mechanical cues further enhances their tissue regeneration potential. Growth factors can promote cell proliferation and differentiation, initiating the regenerative process. Incorporating bioactive molecules into the scaffolds facilitates specific cellular responses, while the application of mechanical cues can influence cell behaviour and tissue organization.
As tissue engineering continues to advance, the integration of functional materials opens up new possibilities in regenerative medicine [21]. These materials provide a platform to address tissue loss, repair injuries, and even fabricate entire organs. Through the development of sophisticated scaffolds and the incorporation of growth-inducing cues, functional materials hold the promise of transforming the way we approach tissue regeneration and heralding a future where the replacement of damaged or diseased tissues becomes an attainable reality.
Biosensors
The emergence of advanced functional materials has ushered in a new era of biosensors, characterized by heightened sensitivity and selectivity for diagnostic applications [22]. Materials such as graphene, carbon nanotubes, and metal nanoparticles have unlocked unique electrical, optical, and electrochemical properties that are harnessed to detect biomarkers and analytes with remarkable precision. These biosensors have revolutionized the field of diagnostics by offering rapid and accurate capabilities, enabling early disease detection and real-time monitoring.
Graphene, a single layer of carbon atoms arranged in a two-dimensional lattice, boasts extraordinary electrical conductivity and a large surface area [23]. These properties make graphene an exceptional platform for immobilizing biomolecules, enhancing the interaction between the target analyte and the biosensor. The sensitivity of graphenebased biosensors allows for the detection of even minuscule concentrations of biomarkers, critical in early diagnosis, when diseases are most treatable.
Carbon nanotubes, on the other hand, are cylindrical structures formed from rolled-up graphene sheets [24]. They exhibit remarkable mechanical strength, high electrical conductivity, and a vast surface area, rendering them highly efficient in capturing specific biomolecules. The unique electronic properties of carbon nanotubes facilitate label-free detection, eliminating the need for additional tagging molecules, thereby simplifying and expediting the diagnostic process.
Metal nanoparticles, such as gold and silver nanoparticles, are renowned for their exceptional optical and electrochemical properties [25]. The Localized Surface Plasmon Resonance (LSPR) of these nanoparticles imparts them with a distinct color change when bound to specific analytes, enabling visual detection of target molecules. Moreover, metal nanoparticles’ electrochemical behavior allows for sensitive and quantitative detection, making them valuable tools for point-of-care diagnostics.
By leveraging the unique properties of these advanced functional materials, biosensors have achieved an unprecedented level of selectivity. Functionalization of the biosensor’s surface with specific biomolecules ensures that only the desired analyte is detected, minimizing interference from other substances. This selectivity is crucial in distinguishing between different diseases and avoiding false positives or negatives, which can have significant consequences in patient care.
The high sensitivity and selectivity of biosensors have far-reaching implications in the realm of diagnostics. These devices empower healthcare professionals to detect diseases at an early stage, even before clinical symptoms manifest, allowing for timely intervention and improved patient outcomes. Additionally, biosensors facilitate real-time monitoring, enabling continuous assessment of a patient’s health status and treatment efficacy, leading to personalized and optimized therapeutic regimens.
Bioimaging Agents
The realm of bioimaging has experienced a transformative revolution with the integration of functional materials, paving the way for high-resolution and real-time visualization of intricate biological systems [26]. Quantum dots, upconversion nanoparticles, and superparamagnetic iron oxide nanoparticles stand as formidable contenders, harnessing unique optical and magnetic properties to enhance imaging contrast and fidelity (Figure 5). These advanced materials enable targeted imaging of specific tissues, cells, or biomolecules, unlocking new avenues for understanding disease progression and fostering the development of personalized treatments [27].
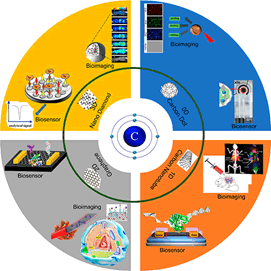
Figure 5: Types of bio-imaging agents
Quantum dots, semiconductor nanoparticles, exhibit remarkable optical properties such as size-tunable emission spectra and exceptional brightness [28]. These attributes make quantum dots ideal fluorescent imaging agents, providing a higher signal-to-noise ratio compared to traditional organic dyes. By engineering quantum dots with specific surface coatings, they can be tailored to target specific biomolecules, offering unprecedented insights into cellular processes and interactions.
Upconversion nanoparticles are a class of materials capable of converting low-energy photons into higher-energy emissions, allowing for deep tissue penetration and reduced background noise. These nanoparticles exhibit anti-Stokes luminescence, where they emit light at shorter wavelengths than the excitation light, which is particularly advantageous for bioimaging applications [29]. Upconversion nanoparticles open up possibilities for in vivo imaging of deeper tissues and dynamic processes within living organisms.
Superparamagnetic iron oxide nanoparticles have magnetic properties that make them suitable for Magnetic Resonance Imaging (MRI) [30]. These nanoparticles act as contrasting agents, enhancing the visibility of specific tissues or organs in MRI scans. Additionally, superparamagnetic nanoparticles can be functionalized to target specific cells or biomarkers, providing crucial information about disease localization and progression.
By leveraging the unique properties of these functional materials, bioimaging has progressed beyond simple anatomical visualization, ushering in a new era of targeted and personalized imaging. Functionalization of imaging agents allows for the selective targeting of disease sites, enabling early detection and accurate monitoring of therapeutic responses. This level of precision helps optimize treatment strategies, minimize side effects, and improve patient outcomes.
The impact of functional materials in bioimaging extends beyond conventional techniques. Advanced imaging modalities, such as multispectral imaging and photoacoustic imaging, are now empowered by the unique properties of quantum dots and upconversion nanoparticles. These techniques provide unprecedented spatial and temporal resolution, enabling visualization of molecular and cellular processes in real-time, a remarkable achievement in the study of living systems.
Bioelectronics, a rapidly evolving field that lies at the interface of biology and electronics, has witnessed significant advancements through the integration of advanced functional materials. These materials play a pivotal role in developing innovative devices and systems that can interact seamlessly with biological systems, enabling novel applications in diagnostics, therapeutics, and bio-sensing. In this section, we explore some of the key bioelectronics applications of advanced functional materials and their transformative impact on healthcare and biomedical research.
Bioelectronic Devices for Neural Interfaces
Advanced functional materials, such as conducting polymers, graphene, and carbon nanotubes, have been instrumental in the development of bioelectronic devices for neural interfaces. These materials possess excellent electrical conductivity and biocompatibility, allowing them to interface with neurons and nerve tissues more effectively. Neural implants based on these materials can facilitate real- time monitoring and stimulation of neural activity, leading to breakthroughs in understanding brain function and offering potential treatments for neurological disorders like Parkinson’s disease and epilepsy.
Flexible and Stretchable Electronics for Wearable Health Monitoring
The advent of advanced flexible and stretchable materials has marked a significant milestone in the evolution of wearable health monitoring devices [31]. Organic electronics and nanocomposites have emerged as transformative elements, reshaping the landscape of health tracking. These materials possess the remarkable ability to conform to the skin’s contours, ushering in a new era of continuous and non-invasive monitoring of vital signs, glucose levels, and various biomarkers. Wearable bioelectronics hold the potential to revolutionize healthcare, offering early disease detection, personalized health tracking, and improved patient outcomes by providing real-time data and facilitating timely interventions (Figure 6) [32].
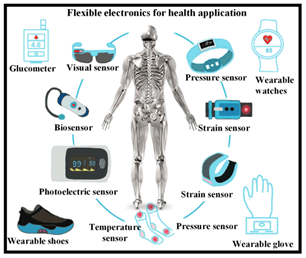
Figure 6: Flexible bioelectronics in health applications
Flexible and stretchable materials provide a comfortable and unobtrusive platform for wearable devices, making them seamlessly integrate with the human body [33]. The pliability of these materials enables wearable health monitors to adhere closely to the skin, ensuring constant contact and reliable data acquisition. This intimate connection allows for the continuous monitoring of essential health parameters, empowering individuals to gain deeper insights into their well-being [34,35].
One of the most significant advantages of wearable health monitoring devices is their potential for early disease detection. By continuously tracking vital signs and biomarkers, these devices can identify subtle changes in health patterns, raising red flags for potential health issues. Timely detection of such changes allows for early intervention, enabling healthcare professionals to intervene before conditions escalate, and empowering patients to take proactive measures in managing their health [35].
Personalized health tracking is another key benefit of wearable bioelectronics. As these devices collect real-time data, they provide individuals with personalized and actionable insights into their health status. Such personalized feedback fosters a better understanding of one’s health habits and encourages healthier lifestyle choices. Moreover, healthcare providers can utilize this data to create tailored treatment plans, ensuring treatments align with each patient’s unique needs.
In the context of chronic conditions like diabetes, wearable health monitors offer continuous glucose monitoring, eliminating the need for frequent finger pricking. This non-invasive approach significantly improves the quality of life for patients, while simultaneously providing a more comprehensive and accurate picture of glucose fluctuations.
Furthermore, wearable health monitoring devices enhance patient outcomes by facilitating timely interventions. The real-time data provided by these devices allows for immediate recognition of any health anomalies, enabling quick responses from healthcare providers. Prompt interventions can prevent complications, reduce hospitalizations, and promote better disease management.
Bioelectronic Sensors for Point-of-Care Diagnostics
Bioelectronic sensors have emerged as powerful tools in the realm of point-of-care diagnostics, thanks to the contributions of advanced functional materials. These sensors, equipped with nanomaterials and surface functionalization techniques, offer unprecedented sensitivity and specificity in detecting disease biomarkers, viruses, and bacteria. Rapid and portable bioelectronic diagnostic devices empower healthcare providers with quick and reliable information, facilitating timely interventions and alleviating the strain on centralized healthcare systems (Figure 7) [36].
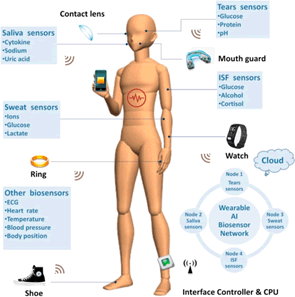
Figure 7: Bio-electronic sensors for point-of-care diagnostics
The integration of nanomaterials in bioelectronic sensors revolutionizes their capabilities, allowing for enhanced detection of biomolecules and pathogens. Nanomaterials, such as quantum dots and gold nanoparticles, possess unique optical and electrical properties that boost the sensitivity of the sensors. These nanomaterials can effectively interact with target analytes, amplifying the sensor’s signal and enabling the detection of low concentrations of disease markers or infectious agents.
Surface functionalization techniques play a crucial role in conferring specificity to bioelectronic sensors. By modifying the sensor’s surface with specific antibodies or aptamers, the sensors become highly selective, enabling the recognition and capture of specific biomolecules or pathogens. This selectivity minimizes false positives and ensures that only the target of interest is detected, enhancing the accuracy and reliability of the diagnostic results.
The compact and portable nature of bioelectronic diagnostic devices makes them ideal for point-of-care applications. These devices can be easily used in diverse settings, including remote areas and resource-limited environments, where immediate and accurate diagnostics are crucial for patient management. Healthcare providers can obtain real- time information at the patient’s bedside, enabling swift decision-making and timely interventions.
Point-of-care bioelectronic sensors also contribute to the early detection of diseases, facilitating timely treatment and better patient outcomes. Rapid and accurate diagnostics allow healthcare professionals to initiate appropriate interventions promptly, preventing the progression of diseases and reducing the overall healthcare burden.
Furthermore, these sensors have proven invaluable in detecting infectious diseases, including viral and bacterial infections. The ability to identify pathogens on-site facilitates immediate isolation and treatment of affected individuals, mitigating the spread of infections and curbing potential outbreaks.
The adoption of bioelectronic sensors for point-of-care diagnostics has transformative implications for healthcare systems. By providing quick and reliable results, these sensors streamline the diagnostic process and reduce the need for centralized testing facilities, thus optimizing healthcare resource allocation. Additionally, the ease of use and portability of these devices empower healthcare workers, enabling them to deliver timely and efficient care to patients.
Bioelectronic Therapeutic Devices
Innovative materials have opened new frontiers in the realm of bioelectronic therapeutic devices, leading to the development of groundbreaking treatments known as bioelectronic medicines and electroceuticals. Electroceuticals utilize electrical signals to modulate neural pathways and biochemical processes, offering targeted and non-pharmacological treatments for a wide range of conditions, such as chronic pain, inflammatory disorders, and metabolic diseases. The fusion of advanced materials with precise engineering has enabled the design of efficient and biocompatible electroceutical implants, paving the way for personalized and minimally invasive therapeutic interventions.
Bioelectronic medicines and electroceuticals represent a paradigm shift in the approach to medical treatments. Unlike traditional pharmaceuticals that rely on chemical agents to influence biological processes, these innovative therapeutic devices use electrical impulses to precisely interact with the body’s neural circuits and biochemical pathways. By directly interfacing with the body’s electrical signals, electroceuticals offer localized and specific therapeutic effects, reducing the risk of systemic side effects associated with many medications.
Advanced materials play a critical role in the development of biocompatible and efficient electroceutical devices. Materials such as biocompatible polymers, conductive nanomaterials, and biodegradable coatings are used in the construction of implantable devices, ensuring compatibility with the body’s tissues and systems. The use of these materials reduces the risk of adverse reactions and improves the longevity of the devices within the body.
One of the key applications of bioelectronic therapeutic devices is the management of chronic pain. Implantable neurostimulators, also known as spinal cord stimulators, deliver electrical pulses to the spinal cord, modulating pain signals and providing relief to patients with chronic pain conditions such as neuropathy or failed back surgery syndrome. This approach offers an alternative to traditional pain management methods, reducing the need for opioid medications and potentially improving patients’ quality of life.
Electroceuticals also show promise in treating inflammatory disorders and metabolic diseases. For example, vagus nerve stimulation, a form of electroceutical therapy, has shown encouraging results in reducing inflammation and improving symptoms in patients with rheumatoid arthritis and inflammatory bowel disease. Additionally, bioelectronic devices that stimulate specific nerves have been investigated for their potential to regulate metabolic processes and treat conditions like obesity and type 2 diabetes.
The precise engineering of these devices allows for personalized treatment plans, tailored to each patient’s specific needs and conditions. Moreover, the minimally invasive nature of many electroceutical implants reduces the risks associated with surgery and shortens recovery times, further enhancing patient outcomes.
Bioelectronics in Tissue Engineering and Regenerative Medicine
Advanced functional materials have emerged as powerful tools in the field of tissue engineering and regenerative medicine, facilitating the creation of biocompatible scaffolds, matrices, and bioactive surfaces. These materials play a pivotal role in guiding cellular behavior, promoting tissue growth, and enhancing the regeneration of damaged tissues and organs. Moreover, the integration of bioelectronic components, such as bioelectrical stimulators, further enhances tissue regeneration and accelerates healing processes.
In tissue engineering, the use of advanced functional materials as scaffolds is crucial for creating a three-dimensional (3D) framework that mimics the extracellular matrix of natural tissues. These materials, often biocompatible and biodegradable, provide a supportive environment for cell adhesion, proliferation, and differentiation. By offering a structure that closely resembles the native tissue, functional materials promote cell attachment and facilitate the growth of new tissue, leading to successful tissue regeneration.
Bioactive surfaces derived from functional materials can be engineered to provide specific cues to guide cellular behavior. For example, the incorporation of growth factors or signaling molecules into the material’s surface can promote targeted cell differentiation or tissue-specific functions. This control over cellular behavior aids in tissue development and helps create complex and functional tissues, such as vascular networks or nerve connections.
Moreover, the versatility of functional materials allows for the design of tissue-specific scaffolds tailored to each regenerative medicine application. For instance, bone tissue engineering may involve the use of materials that mimic the mechanical properties of bone, while cardiac tissue engineering may require materials with electrical conductivity to replicate the heart’s contractile properties.
Incorporating bioelectronic components into tissue engineering constructs takes regenerative medicine to new heights. Bioelectrical stimulators, such as electrical or electromagnetic fields, can be integrated into scaffolds to enhance tissue regeneration and healing. Electrical stimulation has been shown to accelerate wound healing, promote bone regeneration, and improve the formation of vascular networks in engineered tissues.
Additionally, bioelectrical signals can influence cellular behavior, directing cell migration and promoting tissue remodeling. By incorporating bioelectronic components into tissue engineering constructs, researchers can harness the power of electrical signals to optimize tissue regeneration and create tissues that more closely resemble their natural counterparts.
Discussion
Challenges and future directions
Despite the remarkable progress in the field of bioelectronics and advanced functional materials, several challenges need to be addressed to fully exploit their potential in biological applications. These challenges encompass critical aspects such as biocompatibility, long-term stability, scalability, regulatory considerations, and cost-effectiveness. Overcoming these hurdles requires interdisciplinary collaborations and rigorous evaluation of material properties to ensure their safety and efficacy in clinical settings. As the field continues to evolve, future research should focus on designing multifunctional materials with integrated therapeutic and diagnostic capabilities, developing advanced bio-fabrication techniques for complex tissue constructs, and exploring the application of materials in areas such as regenerative medicine and personalized healthcare.
One of the foremost challenges in utilizing advanced functional materials in biological applications is ensuring their biocompatibility. These materials must interact harmoniously with living tissues and cells, minimizing adverse reactions or immune responses. Thorough biocompatibility testing and understanding the mechanisms of material- tissue interactions are essential to mitigate any potential risks.
Long-term stability is crucial, especially for implantable bioelectronic devices. Ensuring that these devices maintain their functionality over extended periods in the harsh environment of the human body remains a challenge. Preventing material degradation, corrosion, or loss of bioactive properties is essential for sustained efficacy.
Advancements in bioelectronics and functional materials must be scalable to meet the growing demand for biomedical applications. Manufacturing processes should be adaptable to produce materials and devices in large quantities without compromising quality and consistency.
Regulatory approvals and compliance with medical device regulations are crucial steps for translating advanced functional materials into clinical practice. Addressing regulatory requirements ensures that these materials meet stringent safety and efficacy standards, instilling confidence among healthcare providers and patients.
The cost of developing and producing advanced functional materials can be a significant barrier to widespread adoption. Research efforts should strive to optimize material synthesis processes and device fabrication techniques to reduce production costs, making these technologies more accessible and affordable for patients and healthcare systems.
Future directions
Researchers should explore the design of multifunctional materials that integrate both therapeutic and diagnostic capabilities. This convergence would enable bioelectronics to act not only as sensing or monitoring devices but also as active therapeutic agents, offering real-time feedback and targeted interventions for precise and personalized healthcare.
Advancements in biofabrication techniques hold tremendous potential for complex tissue engineering. Three-dimensional printing, bioprinting, and tissue engineering approaches should be further developed to create intricate and functional tissue constructs with precise control over architecture and cellular composition.
The application of advanced functional materials in regenerative medicine offers a promising avenue for tissue repair and organ replacement. Research efforts should focus on creating materials that can actively promote tissue regeneration and integration with the host’s biological systems, leading to improved outcomes in regenerative therapies.
The customization of bioelectronics and functional materials for personalized healthcare is a compelling direction for future research. Tailoring materials and devices to individual patient characteristics and medical conditions can enhance treatment efficacy and optimize patient outcomes.
Conclusion
Advanced functional materials have opened new frontiers in healthcare and biomedical research. Custom-tailored materials hold great promise for drug delivery, tissue engineering, biosensing, and bioimaging. Integrating bioelectronics adds an exciting dimension to this progress, enabling personalized and responsive healthcare solutions. However, challenges in safety and biocompatibility must be addressed for successful clinical translation. By fostering collaboration and investment in research, we can unlock the full potential of these technologies and revolutionize healthcare, addressing global health challenges with unprecedented precision and elevating human well-being to new heights.
Acknowledgement
None.
Conflict Of Interest
Authors have no conflict of interest to declare.
References
- A. Kalkal, S. Kumar, P. Kumar, R. Pradhan, M. Willander, et al. Recent advances in 3D printing technologies for wearable (bio)sensors, Addit Manuf, 46(2021):102088.
- T. He, C. Lee, Evolving flexible sensors, wearable and implantable technologies towards BodyNET for advanced healthcare and reinforced life quality, IEEE CASS, 2(2021):702-720.
- A.K. Brooks, S. Chakravarty, M. Ali, V.K. Yadavalli, Kirigami-inspired biodesign for applications in healthcare, Adv Mater, 34(2022):e2109550.
- R. Chelliah, S. Wei, E.B. Daliri, M. Rubab, F. Elahi, et al. Development of nanosensors based intelligent packaging systems: Food quality and medicine, Nanomaterials (Basel), 11(2012):1515.
- Z. Huang, Y. Xu, Y. Cheng, M. Xue, M. Deng, et al. Recent advances in skin-like wearable sensors: Sensor design, health monitoring, and intelligent auxiliary, R Soc Chem, 1(2022):686-708.
- D. Ji, X. Guo, W. Fu, Z. Ding, C. Wang, et al. The marriage of biochemistry and nanotechnology for non-invasive real-time health monitoring, Mater Sci Eng R Rep, 149(2022):100681.
- O.S. Fenton, K.N. Olafson, P.S. Pillai, M.J. Mitchell, R. Langer, Advances in biomaterials for drug delivery, Adv Mater, 30(2018):e1705328.
- K. Chatterjee, S. Sarkar, K.J. Rao, S. Paria, Core/shell nanoparticles in biomedical applications, Adv Colloid Interface Sci, 209(2014):8-39.
- Y. Wang, Z. Li, Q. Hu, Emerging self-regulated micro/nano drug delivery devices: A step forward towards intelligent diagnosis and therapy, Nano Today, 38(2021):101127.
- N. Bardhan, Nanomaterials in diagnostics, imaging and delivery: Applications from COVID-19 to Cancer, MRS Commun, 12(2022):1119-1139.
- F. Ullah, M.B. Othman, F. Javed, Z. Ahmad, H.M. Akil, Classification, processing and application of hydrogels: A review, Mater Sci Eng C Mater Biol Appl, 57(2015):414-33.
- M. Rizwan, R. Yahya, A. Hassan, M. Yar, A.D. Azzahari, et al. pH sensitive hydrogels in drug delivery: Brief history, properties, swelling, and release mechanism, material selection and applications, Polymers (Basel), 9(2017):137.
- Y. Ouyang, J. Zhao, S. Wang, Multifunctional hydrogels based on chitosan, hyaluronic acid and other biological macromolecules for the treatment of inflammatory bowel disease: A review, Int J Biol Macromol, 227(2023):505-523.
- J. Wankar, N.G. Kotla, S. Gera, S. Rasala, A. Pandit, et al. Recent advances in host-guest self-assembled cyclodextrin carriers: Implications for responsive drug delivery and biomedical engineering.
- S. Caddeo, M. Boffito, S. Sartori, Tissue engineering approaches in the design of healthy and pathological in vitro tissue models, Front Bioeng Biotechnol, 5(2017):40.
- M. Alonzo, F.A. Primo, S.A. Kumar, J.A. Mudloff, E. Dominguez, et al. Bone tissue engineering techniques, advances and scaffolds for treatment of bone defects, Curr Opin Biomed Eng, 17(2021):100248.
- M. Rahmati, D.K. Mills, A.M. Urbanska, M.R. Saeb, J.R. Venugopal, et al. Electrospinning for tissue engineering applications, Prog Mater Sci, 117(2021):100721.
- Q. Chai, Y. Jiao, X. Yu, Hydrogels for biomedical applications: Their characteristics and the mechanisms behind them, Gels, 3(2017):6.
- Y. Ikada, Tissue engineering: Fundamentals and applications, 2011.
- E.C. Wu, S. Zhang, C.A.E. Hauser, Self-assembling peptides as cell-interactive scaffolds, Adv Funct Mater, 22(2012):456-468.
- A. Maleki, M. Ghomi, N. Nikfarjam, M. Akbari, E. Sharifi, et al. Biomedical applications of MXene-integrated composites: Regenerative medicine, infection therapy, cancer treatment, and biosensing, Adv Funct Mater, 32(2022):2203430.
- J. Sengupta, C.M. Hussain, CNT and graphene-based transistor biosensors for cancer detection: A review, Biomolecules, 13(2023):1024.
- A. Sharma, A. Singh, V. Gupta, S. Arya, Advancements and future prospects of wearable sensing technology for healthcare applications, R Soc Chem, 1(2022):387-404.
- R. Hirlekar, M. Yamagar, H. Garse, M. Vij, V. Kadam, Carbon nanotubes and its applications: A review, Asian J Pharm Clin Res, 2(2009):17-27.
- H. Kang, J.T. Buchman, R.S. Rodriguez, H.L. Ring, J. He, et al. Stabilization of silver and gold nanoparticles: Preservation and improvement of plasmonic functionalities, Chem Rev, 119(2019): 664-699.
- J. Wei, Y. Liu, J. Yu, L. Chen, M. Luo, et al. Conjugated polymers: Optical toolbox for bioimaging and cancer therapy, Small, 17(2021):2103127.
- B. Pelaz, C. Alexiou, R.A. Alvarez-Puebla, F. Alves, A.M. Andrews, et al. Diverse applications of nanomedicine, ACS Nano, 11(2017):2313-2381.
- A.M. Wagner, J.M. Knipe, G. Orive, N.A. Peppas, Quantum dots in biomedical applications, Acta Biomater, 94(2019):44-63.
- F. Wang, D. Banerjee, Y. Liu, X. Chen, X. Liu, Upconversion nanoparticles in biological labeling, imaging, and therapy, Analyst, 135(2010):1839-54.
- C. Rumenapp, B. Gleich, A. Haase, Magnetic nanoparticles in magnetic resonance imaging and diagnostics, Pharm Res, 29(2012):1165-1179.
- M. Baharfar, M. Rahbar, M. Tajik, G. Liu, Engineering strategies for enhancing the performance of electrochemical paper-based analytical devices, Biosens Bioelectron, 167(2020):112506.
- M. Civas, M. Kuscu, O. Cetinkaya, B.E. Ortlek, O.B. Akan, Graphene and related materials for the internet of bio-nano things, cs ET, (2023).
- J. Yin, R. Hinchet, H. Shea, C. Majidi, Wearable soft technologies for haptic sensing and feedback, 31(2021):2007428.
- D. Marculescu, R. Marculescu, N.H. Zamora, P. Stanley-Marbell, P.K. Khosla, et al. Electronic textiles: A platform for pervasive computing, IEEE, 91(2003):1995-2018.
- J. Min, J. Tu, C. Xu, H. Lukas, S. Shin, Skin-interfaced wearable sweat sensors for precision medicine, Dis Mon, 123(2023):5049-5138.
- R.S. Hazra, M.R. Hasan Khan, N. Kale, T. Tanha, J. Khandare, et al. Bioinspired materials for wearable devices and point-of-care testing of cancer, ACS Biomater Sci Eng, 9(2017):2103-2128.
Copyright: © 2023 Iqra Qayyum, et al. This is an open access article distributed under the terms of the Creative Commons Attribution License, which permits unrestricted use, distribution, and reproduction in any medium, provided the original work is properly cited.